During the COVID-19 pandemic, many phrases like “test and trace” or “test and release” were very common not only in healthcare facilities but also in almost all publicly accessible areas (Fig. 1). While these were new to many, it actually underpinned a decades-old technology that enabled rapid identification and/or quantification of analytes that are biologically relevant. Such technology was key to allow the infection rate to be curbed by following self-isolation and border control procedures.
During the COVID-19 outbreak, the ability to analyse samples quickly was critical to reduce turnaround time and prevent further spread of infection. Initially, samples were only analysed in central testing facilities using the well-established nucleic acid assay called known as polymerase-chain reaction, or PCR, with a turnaround time between a few hours to a few days along with the need for highly trained operators and expensive equipment. Later, other convenient alternatives to this strategy started to emerge where untrained users could simply analyse their nasopharyngeal swabs at home in a few minutes using a lateral flow device (LFD) or rapid antigen test (RAT).
This transition from laboratory-based testing to rapid testing was not possible without the accumulating knowledge and research that supported the development of biosensors. A biosensor is a device that can report on a pathophysiological condition using a simple and easy to use device that allows untrained users (or at least users with basic training) to test their own samples at the point-of-need, either at home or in a healthcare facility. These devices reduce the need for sample transport to central laboratories and allow swift management of health conditions by providing quick and reliable information enabling accurate clinical assessments.1
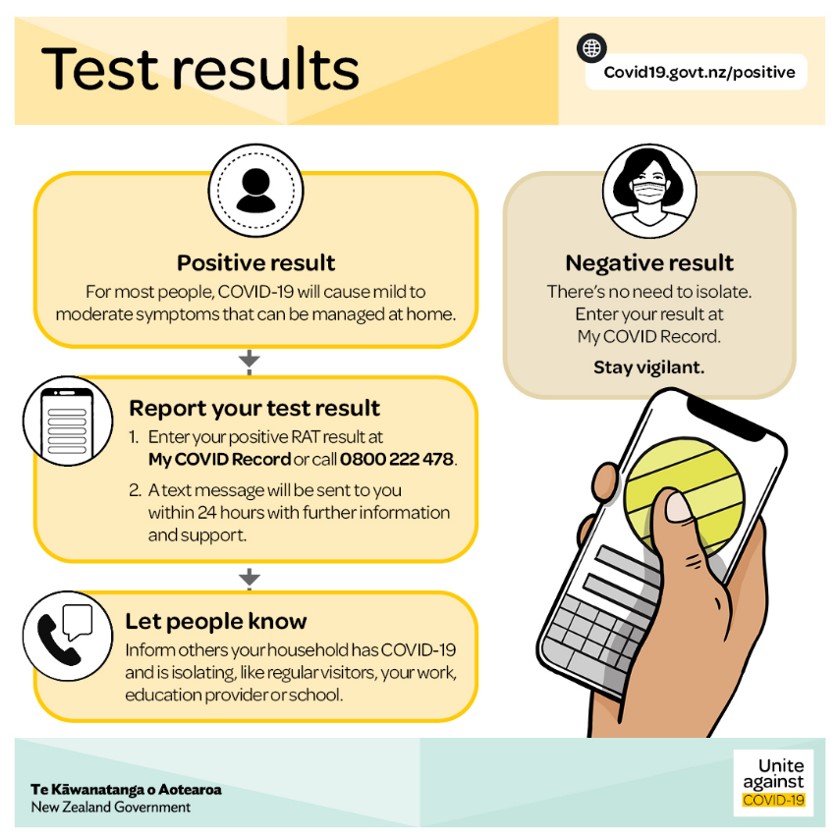
One of the first commercially available (and most successful) biosensor was the glucose sensor or glucometer, widely used to measure the levels of glucose in blood collected from a finger prick. While insulin discovery was one of the most significant, life-changing events for millions of diabetic patients, its significance was not fully realised until these glucometers were invented.2
The introduction of glucose home testing kits in the 1970s
was a landmark change where the concept of self-monitoring of blood glucose (SMBG) became the standard for diabetic care. These glucose sensors, built on electrochemical platforms, enabled a much more accurate glucose analysis with a quick response and high sensitivity.
Initially, glucose levels used to be measured in urine samples using Benedict’s reagent invented in 1908. Benedict’s reagent is a copper-based mixture to detect reducing sugars that requires a cumbersome heating procedure and is susceptible to interferences from many reducing chemicals.3 The introduction of glucose home testing kits in the 1970s was a landmark change where the concept of self-monitoring of blood glucose (SMBG) became the standard for diabetic care. These glucose sensors, built on electrochemical platforms, enabled a much more accurate glucose analysis with a quick response and high sensitivity. Glucose sensors did not only provide better management of diabetes, but also initiated a new field of research focusing on biosensor development and investigation of their use in biomedical applications, the pharmaceutical industry, food quality control and drug and border control.
Biosensors for biomedical applications
The development of biosensors for biomedical applications spans a range of disease diagnostics and point-of-care (POC) health monitoring. With the huge leap in drug development targeting specific biochemical pathways underlying different diseases, there was a pressing need for more precise and accurate diagnostics at the POC - also known as personalised or precision medicine. The genetic and physiological variations between patients are known to be decisive in determining the best course of treatment for a specific condition.
Biosensors targeting specific biomarkers (protein, nucleic acid or metabolites) abnormally expressed during disease progression, can accurately identify disease stage and report on response to therapeutic regimes. Do to their sensitivity, ease of use and fast response, biosensors can support early detection of diseases, personalised treatment and monitoring of disease progression as well as guide personalised therapeutic interventions. To highlight the biomedical applications of biosensors we herein demonstrate the significance of using biosensors in the detection of two leading causes of death; cardiovascular diseases (CVDs) and cancer.
Cardiovascular diseases are the leading cause of death worldwide accounting for more than 31% of all deaths in 2016.4 Patients admitted to emergency rooms with chest pain are usually examined using diagnostic electrocardiogram (ECG). Half of CVD patients show normal or no diagnostic electrocardiograms complicating the accurate and fast diagnosis of the condition.5 Analysis of biomarkers in serum is a more accurate and sensitive alternative but requires central laboratory testing which, in some cases (especially in areas with limited resources), can delay life-saving treatments. Biosensors can bridge this gap by enabling a fast, sensitive analysis of CVD biomarkers at the POC where analysis in an ambulance or emergency room support early-stage diagnosis which allows successful treatment and recovery of CVD patients.
An array of biomarkers has been identified and validated for different CVD conditions including cardiac troponin I (cTnI) and creatine kinase MB subform (CK-MB) for acute myocardial infarction; B-type natriuretic peptide (BNP) for acute coronary syndromes or heart failure; C-reactive protein (CRP) and tumour necrosis factor alpha (TNF-α) as markers for inflammation and cardiac risk factors. Several commercial biosensors are now available for such applications; for example the Minicare© system (Philips) for the quantitation of cTnI and BNP in serum, TriageTrue© (Quidel) for detection of cTnI and i-STAT© (Abbott) to determine BNP and cTnI.6 Although these systems offer life-saving tools that assist clinicians in the emergency room, there is a need for better sensing performance in terms of cost, time and sensitivity. For example, most commercial systems can detect cTnI down to 1 ng/mL while its normal range in blood is between 0-0.04 ng/mL (well below the range detectable by commercial sensors), underpinning the need for more sensitive techniques to detect concentrations down to a few pg/mL.7
On the other hand, cancer as the second leading cause of death worldwide is heterogenous in nature and its diagnosis is as challenging as its treatment. The ability to diagnose cancer at an early stage before presentation of symptoms is associated with effective and successful treatment. Current diagnostic strategies rely mainly on the development of symptoms and imaging techniques that usually identify cancer after development of significant cancerous lesions (tissue), limiting the efficacy of treatment and increasing the risk of spreading cancer to other parts of the body, known as metastasis.
Recent genetic, proteomic and metabolomic analyses have correlated the abnormal expression of several biomarkers to different types of cancer. These biomarkers, advantageously, can identify cancer at a very early stage well before the development of any symptoms. Assessing the expression of these biomarkers supports cancer screening programmes that enable efficient and successful therapeutic interventions.8
The most prominent examples of these programmes are breast and prostate cancer screening adopted in many healthcare systems. Testing serum levels of prostate specific antigen (PSA) and examining BRCA1 and BRCA2 gene variations as predisposition signs of prostate and breast cancer respectively allow identification of more susceptible individuals initiating, personalised health-monitoring programmes. Alas, current screening techniques suffer from low specificity, resulting in false positive diagnoses which puts many patients under psychological stress while adding more cost and pressure on healthcare systems. While there are no commercially available cancer biosensors, research in this field can allow highly sensitive cancer diagnosis with good sensitivity. In addition, biosensors can offer an accessible tool for physicians to tailor treatment regimes and dosing according to individual responses to administered drugs.
Interestingly, recent evidence supports the use of biomarker panels for cancer identification rather than less-reliable single biomarker assays. For example, a panel of 8 biomarkers (including PSA, vascular endothelial growth factor-D (VEGF-D), gene fusion proteins ETS related gene (ERG), Golgi membrane protein 1 (GOLM-1), pigment epithelial derived factor (PEDF), insulin-like growth factor-1 (IGF-1), insulin-like growth factor binding protein-3 (IGFPB-3) and serum monocyte differentiation antigen CD-14 (CD-14)) can accurately predict aggressive vs indolent forms of prostate cancer. This test was performed in an electrochemical microfluidic system that can be easily integrated into a commercial platform (Fig. 2).9

Other endeavours investigating system automation and integration of multiple steps demonstrated biosensors’ ability to detect cell-bound biomarkers for cancer metastasis. The system was built on a 3D-printed microfluidic chip that can extract cell-membrane bound protein, desmoglein 3 (DSG3), a metastatic biomarker for oral cancer. The extracted DSG3 was then quantified within the same microfluidic chip using a well-established chemiluminescence assay with very high sensitivity down to fg/mL (Fig. 2). Such a system allows clinicians to decide on a specific course of treatment since a high level of DSG3 indicates the presence of metastatic cancerous tissue that require dissection.10 These examples highlight the potential capabilities of using biosensors in CVD and cancer diagnosis and demonstrate the advantages of integrating them into the healthcare system workflow where it can allow better, more efficient treatment and improve quality of care while reducing costs and time spent in hospitals.
Biosensor applications in the pharmaceutical industry
Biosensors, by virtue of their ability to monitor events in real-time, offer an excellent tool to evaluate different processes within pharmaceutical industries, helping companies meet production and compliance goals. Biosensor tasks can vary from simple functions like monitoring oxygen and pH to quantifying drugs for quality control, assessing sterility, detecting common impurities or contaminants and running bioavailability and pharmacokinetic studies. These functions can be easily achieved by integrating optical or electrochemical sensors within a production line to guide scale up or scale down activities. A well-established example of integrating biosensors within production lines is the use of multiple infrared sensors to monitor the homogeneity of active ingredients within a powder blend during tablet manufacturing. This enables semi-automated control on the blend end-point to ensure tablet content uniformity.11
The application of biosensors in the pharmaceutical field has been further highlighted by the rapid evolution of immunotherapeutic agents which usually require expensive, lengthy and laborious analysis. The increased interest in using immunomodulators or therapeutic vaccines for treatment of cancer or other conditions requires efficient tools for their quantitation and evaluation of their functionality. The quality control process of immunotherapies is not limited to just quantitative analysis but should also report on their pharmacological and therapeutic effect including multi-parametric analysis of cell function, real-time detection of secreted signals and facile retrieval of cells of interest (Fig. 3).12
Currently, polychromatic flow cytometry is the main technique applied for monitoring immunotherapeutic agents, both in preclinical tumour immunology and in cancer immunotherapy trials. While polychromic flow cytometry offers the ability to detect multiple target analytes simultaneously, it is expensive and requires very sophisticated equipment, highly trained operators and complex data for in vitro analysis to study cells during manufacturing and testing processes. This is reflected in the high cost of immunotherapy development that requires prolonged testing and evaluation procedures.13
The use of biosensors in such process can drastically reduce the associated cost of immunotherapy development and evaluation, especially with the development of cell-based biosensors that can provide real-time quantitative response to immunotherapeutic agents. This has been extensively studied using label-free optical biosensors, particularly those using surface plasmon resonance where an evanescent wave generated at the plasmonic active interface (usually gold) interacts with a specially engineered incident beam of light and responds sensitively to changes close to the interface. These sensors have been applied to the detection and quantitation of secreted proteins, intracellular changes (known as cytosolic dynamic mass redistribution (DMR)) and tumour and anti-tumour cells in analysed samples (Fig. 3).14
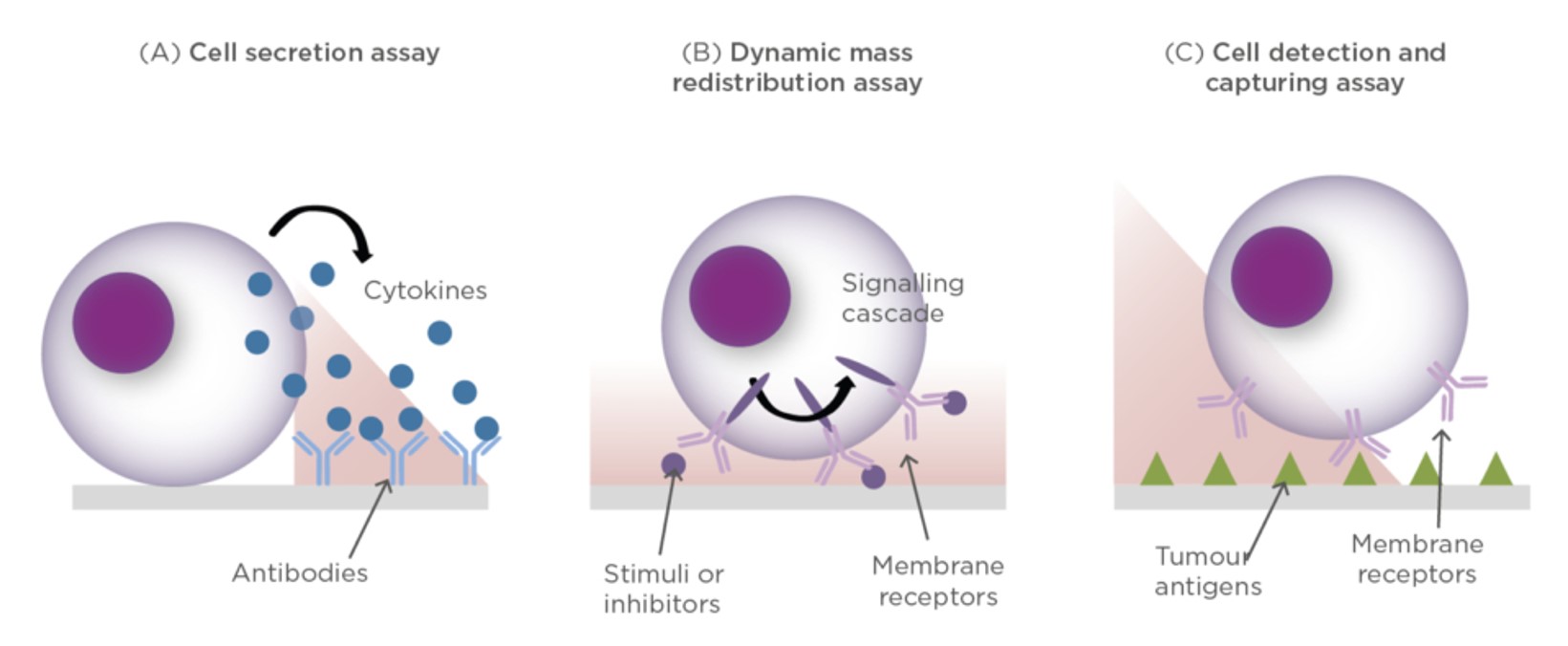
Food quality control
The application of biosensors in the food processing industry is gaining a lot of momentum, especially with the immense need for energy saving and minimising the environmental impact of food production and waste management (Fig. 4). As previously mentioned, biosensors offer rapid and sensitive real-time monitoring of specific target analytes enabling fast detection of the presence of hazardous byproducts or viable cells during food processing. This information is critical in automating food processing and can reduce cost and time as such tighter quality control measures reduce waste, improve food production quality and sustainability.

Biosensors ranging from pH probes to sensing tags integrated within food packaging allow monitoring of storage and transport conditions. In addition, biosensors have been widely applied in the detection of food contaminants like pesticides, toxins or foodborne pathogens. A plethora of commercial food biosensors have been introduced into the food industry over the past two decades; for example RIDACUBE SCAN by r-biopharm© for onsite monitoring of lactic and acetic acid, sugars, ascorbic acid and alcohol; VitaFast© systems for vitamins; BIOFISH 300 from BIOLAN for sulfite detection and PremiTest from r-biopharm for the detection of antibiotic residues.15 Unfortunately, there is still a huge gap between market need and available biosensors due to the increasing range of food contaminants, high rate of food counterfeiting and adulteration and the uncontrolled use of antibiotics and pesticides.
Future scope
The examples discussed in this article are by no means an exhaustive list of biosensors’ applications. Rather they highlight the benefits the biosensor industry can bring to many fields ranging from healthcare affordability to food safety. Biosensors can provide critical information quickly and specifically to allow swift decision-making based on real-time analyses. While current technology has achieved many milestones required for efficient biosensing applications, there are still many areas where further development is required.
For example, biosensor fabrication has emerged from high-cost sophisticated machinery to using paper-based and 3D printed chips for both prototyping or mass-production. Using 3D printing to manufacture biosensors can enable new levels of scalability and affordability to be reached, capitalising on the availability of low-cost desktop 3D printers. This can enable production of previously difficult-to-manufacture architectures that can further improve sensors’ performance and capabilities. Additionally, the advances in engineered micro-components that can be integrated in electrochemical, optical or spectro-electrochemical sensors can allow further miniaturisation without compromising sensor sensitivity.
The use of portable hand-held potentiostats, complementary metal oxide semiconductor (CMOS) sensors, LEDs and fiber optics can allow the production of next-generation biosensors that can achieve benchmark performance of laboratory equipment within a small, low-cost device.
The development of cell-based responsive biosensors, tissue-on-chip and organ-on-chip is another area of development where biosensors can pave the way for fast drug development and validation. The introduction of new sensing platforms that improve sensor sensitivity and reduce the number of steps typically required to run an immunoassay is an equally important area of development. Biosensors with high sensitivity that can detect ultra-low concentrations of target species allow discovery of new biomarkers that are usually missed with current analytical tools. Additionally, biosensors can support studies required to validate panels of biomarkers to evaluate different diseases or physiological conditions. These can afford accurate non-invasive tools for disease diagnostics, improve the quality of healthcare systems, ensure tighter quality control measures in food and pharmaceutical industries and strengthen the current inventory of tools required for preparedness for future healthcare crises or pandemics.
References
1. Sharafeldin, M.; Davis, J. J. Anal. Chem. 2021, 93, 184-197.
2. Yoo, E. H.; Lee, S. Y. Sensors 2010, 10, 4558-4576.
3. Hirsch, I. B. Introduction: History of Glucose Monitoring. In Role of Continuous Glucose Monitoring in Diabetes Treatment, American Diabetes Association, 2018.
4. Gachpazan, M.; Mohammadinejad, A.; Saeidinia, A.; Rahimi, H. R.; Ghayour-Mobarhan, M.; Vakilian, F.; Rezayi, M. Anal. Bioanal. Chem. 2021, 413, 5949-5967.
5. Qureshi, A.; Gurbuz, Y.; Niazi, J. H. Sens. Actuators B Chem. 2012, 171-172, 62-76.
6. Savonnet, M.; Rolland, T.; Cubizolles, M.; Roupioz, Y.; Buhot, A. J. Pharm. Biomed. Anal. 2021, 194, 113777.
7. Mahajan, V. S.; Jarolim, P. Circulation 2011, 124, 2350-2354.
8. Sharafeldin, M.; Kadimisetty, K.; Bhalerao, K. S.; Chen, T.; Rusling, J. F. Sensors 2020, 20, 4514.
9. Jones, A. L.; Dhanapala, L.; Baldo, T. A.; Sharafeldin, M.; Krause, C. E.; Shen, M.; Moghaddam, S.; Faria, R. C.; Dey, D. K.; Watson, R. W.; et al. Anal. Chem. 2021, 93, 1059-1067.
10. Sharafeldin, M.; Chen, T.; Ozkaya, G. U.; Choudhary, D.; Molinolo, A. A.; Gutkind, J. S.; Rusling, J. F. Biosens. Bioelectron. 2021, 171, 112681.
11. Igne, B.; Talwar, S.; Drennen, J. K.; Anderson, C. A. J. Pharm. Innov. 2013, 8, 45-55.
12. Gouttefangeas, C.; Walter, S.; Welters, M. J. P.; Ottensmeier, C.; van der Burg, S. H.; Chan, C. Flow Cytometry in Cancer Immunotherapy: Applications, Quality Assurance, and Future. In Cancer Immunology: A Translational Medicine Context, Rezaei, N. Ed.; Springer International Publishing, 2020; pp 761-783.
13. Sewell, W. A.; Smith, S. A. Pathology 2011, 43, 580-591.
14. Soler, M.; Lechuga, L. M. Eur. Med. J. 2019, 4, 124-132.
15. Di Nardo, F.; Anfossi, L. Chapter Eight - Commercial biosensors for detection of food additives, contaminants, and pathogens. In Commercial Biosensors and Their Applications, Sezgintürk, M. K. Ed.; Elsevier, 2020; pp 183-215.