Cisplatin (1) is a simple square–planar metal complex which contains two labile chlorine atoms and two ammonia molecules coordinated to a central platinum(II) atom in a cis configuration (Fig. 1). While cisplatin was discovered in 1845, it was not until its discovery as a cytotoxic agent by Rosenberg et al. in 19651 that the complex gained prominence, soon becoming the premier metallic based pharmaceutical drug in the treatment of a variety of cancers. In 1990, the implementation of cisplatin as a medication was linked to an increased cancer cure rate of 75%, up from 10% prior to implementation of the drug.2
Cisplatin works in part by binding to guanine and adenine residues on DNA,3 therefore inhibiting its replication3 (Fig. 1). This mode of action primarily targets fast replicating cells such as cancer cells but also the stomach lining and hair follicles, leading to the common side effects of hair loss and nausea. Other common side effects include bone marrow suppression, kidney damage, numbness, electrolyte issues and heart disease.4 Cisplatin has also been shown to harm the developing fetus during pregnancy.
While potent toxicity is advantageous for the treatment of cancer, the non-selective nature of cisplatin has created a desire for the development of the next generation of metallodrugs. Research in this area now spans the entire transition metal block of the periodic table, in the hope of finding treatments not only for cancer but other diseases as well.

This review will give a brief overview on metallodrugs, including their history and current research areas.
Versatility of metallodrugs
Metallodrugs contain three main features of tailorability: the ligands coordinating the metal, the metal centre itself and its corresponding oxidation state. These three features contribute to the vast degree of variation and versatility of the compounds, making them much more tailorable than their organic counterparts. They also provide easy modulation for specific targeting and delivery mechanisms.
Ligands
Ligands are arguably the most important aspect of the metallodrug structure. The coordination mode, the geometry and the chemical properties of the ligand dictate not only the macro physicochemical properties of the resulting complex, but also the mode of action and potential biological targets.5 Ligands also provide the simplest and most robust route for tailoring the chemistry of the resulting complex. The case of cis- and trans-platin is a good example of the importance of the ligand’s geometry. Both isomers contain labile chlorine atoms which can undergo exchange within the cell, allowing the metal to coordinate as a bridge to the guanine residues on DNA. The trans isomer, however, is limited by steric constraint, preventing it from creating bridged complexation and therefore lowering its overall toxicity to sub therapeutically active concentrations.6-7
Metals
Due to the success of initial platinum based compounds, the majority of early examples of metallodrugs are based on platinum(II) with the primary mode of action being based on

DNA binding. These complexes contain a high amount of unfavourable side effects which in most cases outweigh the high efficiency and cytotoxicity of the drugs.8 Due to this low selectivity, non-platinum metals have been extensively researched of late in attempts to find more selective acting drugs with reduced side effects while maintaining cytotoxic efficiency.
Commonly studied metals include Ti, Fe, Cu, Ru, Pd, Ag, Os, Ir and Au. Chief among these is ruthenium since the discovery of the biological activity of the ruthenium complexes RAPTA-C (2), NAMI-A (3) and BOLD-100 (4) among others (Fig. 2).9-11
Oxidation state
The oxidation state of the central metal atom plays an important role in not only the types of ligands that can coordinate to the metal but also in the geometry they occupy. Both aspects have significant effects on the chemistry of the complex, especially when considering the importance of the ligands, as discussed previously. The ability of transition metals to undergo redox related reactions between stable oxidation states has prompted research into metallic based prodrugs.12-13 These prodrugs are able to be activated by redox processes within the cell or by mechanisms specific to the target which allows the switching between oxidation states and therefore the changing of the coordination environment.14 The ultimate goal of manipulating the oxidation state is to increase the overall selectivity of the drug or by increasing its variation by the implementation of new ligand binding sites.15

Platinum(II) and platinum(IV) based metallodrugs
Besides cisplatin, other early platinum(II) based metallodrugs such as carboplatin16 (5) and oxaliplatin17 (6) (Fig. 3) have seen clinical success against ovarian cancer and colon cancer respectively. Both carboplatin and oxaliplatin are considered second generation platinum drugs,18 which have aimed to improve toxicological profiles and are often used in combination therapy with other drugs.
While slightly more selective than cisplatin and with fewer side effects, these second generation drugs suffer from increased drug resistance, which third generation drugs aim to overcome.16 The key structural characteristics of platinum(II) compounds are the archetypal square-planar structure, the cis configuration of the labile ligands and the presence of at least one N-H group; the latter is important due to the hydrogen-bond donor properties.7 Platinum(IV) is also an attractive contender for metallodrugs19 as they often offer greater stability allowing for a great proportion of the drug to reach the target intact. Satraplatin20 (7) (Fig. 4), a platinum(IV) metallodrug, has been known since 1993 but is yet to receive FDA approval.

More recently, platinum(IV) compounds have been investigated as potential prodrugs.12,21 Traditionally, platinum(IV) prodrugs are synthesised from their platinum(II) precursors by oxidative reactions followed by additional ligands coordinating to the newly available axial positions on the octahedral platinum metal centre.

These newly introduced ligands are often seen as the “payload” which are released upon the inevitable reduction of platinum(IV) to platinum(II) (Fig. 5). This process has the added benefit of stabilising the complex until it has reached the target as well as introducing the payload ligands which increase the diversity of treatment.
Non platinum metallodrugs
There is no doubt that platinum based metallodrugs have had a profound impact on the treatment of cancer by chemotherapy, yet they possess significant drawbacks. To overcome these shortcomings, non-platinum based compounds are being investigated22 and are already in use or in clinical trials.23
Ruthenium(III)
Ruthenium octahedral complexes are a potential candidate for non-platinum drugs. Known examples include NAMI-A and KP1019 which are both currently in clinical trials.24 Ruthenium piano stool structures (8) (Fig. 6) are of considerable interest with many reviews being conducted on their applications as metallodrugs.25-27
For example, the RAPTA class of metallodrugs (Fig. 2),28 bearing arene “seats”, water soluble phosphines and labile chlorines, provide extensive room for variation.
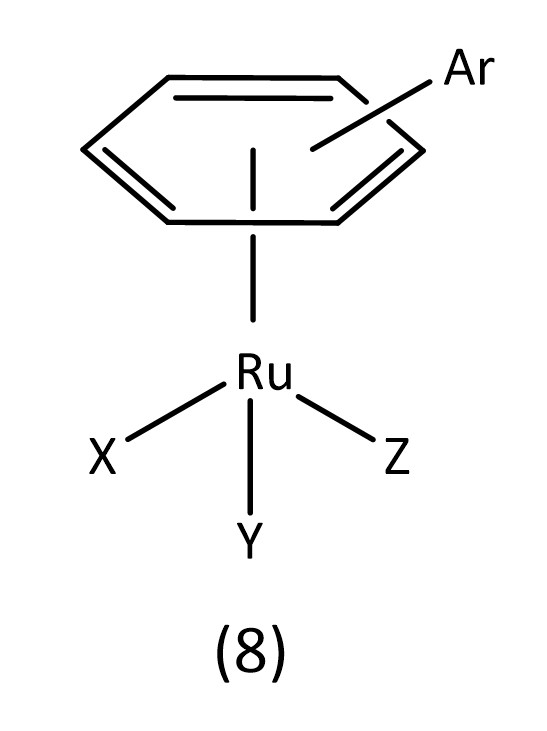
"Ruthenium metallodrugs are attractive alternatives to platinum due to the different modes of action against cancer cells."
Ruthenium metallodrugs are attractive alternatives to platinum due to the different modes of action against cancer cells. While ruthenium based compounds have been shown to bind to DNA,28 they have also been shown to interact with proteins,29 opening avenues for future drug discovery.

Gold(I)
Gold compounds are also attractive avenues for metallodrug research. Auranofin (9) (Fig. 7) is a clinical drug used in the treatment of rheumatoid arthritis30 and has also shown promising results in the treatment of HIV, cancer31 and tuberculosis. Most recently it has shown potential to inhibit the SARS-CoV-2 virus replication.32
Other gold phosphine complexes have also been examined, such as the chlorine analogue of auranofin, chloro(triethylphosphine)gold33 (10) and also the gold complex of bis(diphenylphosphino)ethane34 ([Au(dppe)2]+Cl-) (11) (Fig. 7). It is thought that these gold complexes act on DNA, RNA and protein synthesis,35 with some studies suggesting mitochondria or enzymes to be the target.36 Gold(I) as well as gold(III) continue to show potential for the development of novel metallodrugs.
Alternative delivery mechanisms
Due to the large degree of structural variation that metallodrugs possess, many drugs have been produced thus far for the treatment for a large array of diseases. However, as these metallodrugs suffer from poor targeting, poor bioaccumulation or unpleasant side effects, the most obvious remedy to these drawbacks is the alteration of metallodrug structure. This will not only give the opportunity to impart the desired properties, but it will also allow for the maintaining of the desired efficiency and cytotoxic effects. An alternative approach is to use compounds or structural motifs that are already proven to be effective with a different delivery system which can bypass the issue of poor targeting and potentially reduce unwanted side effects.
Lipid based nanoparticles
One such example of an alternative delivery mechanism is the employment of lipid based nanoparticles,37-38 a technique that has most recently been used for the transport of mRNA in COVID-19 vaccines. Liposomes, consisting of bilayer spherical vesicles comprised of phospholipids, hold an internal hydrophilic core. This core can be used to contain payloads such as metallodrugs.
These lipid nanoparticles have the advantage of biocompatibility allowing the drug to easily traverse the body to reach the targeted area while keeping the compound inside stable.
It also allows for the transport of more hydrophobic drugs, increasing the range of diversity. Some lipid based encapsulation methods have already seen some clinical success, such as the cancer drug liposome-encapsulated doxorubicin.39

Smart delivery systems
Another potential method for overcoming the limitations of metallodrugs is the employment of smart delivery systems. This method involves the use of a carrier molecule, such as a peptide, protein, antibody or hormone.40 These carrier molecules are usually attached to the target drug by a linkage molecule which is separated by external stimuli, such as ion concentration changes, protein docking or even by ultrasound (Fig. 8). While proven success is limited, the use of smart delivery systems for the transport of metallodrugs is in its early stages of research and represents a promising direction for the field.
Conclusions
Metallodrugs continue to be an active and important research field in inorganic chemistry. These versatile metal complexes have the potential for an array of biological functions, with compounds such as cisplatin demonstrating their importance in medicine. While metallodrugs suffer from numerous downsides such as unpleasant side effects and poor selectivity, their versatility allows for active research to overcome these challenges.
References
1. Rosenberg, B.; Van Camp, L.; Krigas, T. Nature 1965, 205 (4972), 698-699.
2. Einhorn, L.H. J. Clin. Oncol. 1990, 8 (11), 1777-1781.
3. Siddik, Z.H. Oncogene 2003, 22 (47), 7265-7279.
4. Oun, R.; Moussa, Y.E.; Wheate, N.J. Dalton Trans. 2018, 47 (19), 6645-6653.
5. Haas, K.L.; Franz, K.J. Chem. Rev. 2009, 109 (10), 4921-4960.
6. Nafisi, S.; Norouzi, Z. DNA Cell Biol. 2009, 28 (9), 469-477.
7. Kostova, I. Rec. Pat. Anti-Cancer Drug Disc. 2006, 1 (1), 1-22.
8. Ott, I.; Gust, R. Int. J. Pharm. Med. Chem. 2007, 340 (3), 117-126.
9. Rausch, M.; Dyson, P.J.; Nowak-Sliwinska, P. Adv. Therap. 2019, 2 (9), 1900042.
10. Kostova, I. Curr. Med. Chem. 2006, 13 (9), 1085-1107.
11. Neuditschko, B.; Legin, A.A.; Baier, D.; Schintlmeister, A.; Reipert, S.; Wagner, M.; Keppler, B. K.; Berger, W.; Meier-Menches, S.M.; Gerner, C. Angew. Chemie Int. Ed. 2021, 60 (10), 5063-5068.
12. Xu, Z.; Wang, Z.; Deng, Z.; Zhu, G. Coord. Chem. Rev. 2021, 442, 213991.
13. Zhao, Z.; Zhang, X.; Li, C.-e.; Chen, T. Biomaterials 2019, 192, 579-589.
14. Yao, H.; Chen, S.; Deng, Z.; Tse, M.-K.; Matsuda, Y.; Zhu, G. Inorg. Chem. 2020, 59 (16), 11823-11833.
15. Gibson, D. J. Inorg. Biochem. 2021, 217, 111353.
16. Vasconcellos, V.F.; Marta, G.N.; da Silva, E.M.; Gois, A.F.; de Castria, T.B.; Riera, R. Cochrane Database System. Rev. 2020, 1.
17. Xu, Z.; Chan, H.M.; Li, C.; Wang, Z.; Tse, M.-K.; Tong, Z.; Zhu, G. Inorg. Chem. 2018, 57 (14), 8227-8235.
18. Rottenberg, S.; Disler, C.; Perego, P. Nature Rev. Cancer 2021, 21 (1), 37-50.
19. Gibson, D. J. Inorg. Biochem. 2019, 191, 77-84.
20. Alassadi, S.; Pisani, M.J.; Wheate, N.J. Dalton Trans. 2022, 51 (29), 10835-10846.
21. Li, X.; Liu, Y.; Tian, H. Bioinorg. Chem. Appl. 2018, 2018.
22. Domínguez-Martís, E.M.; Mosteiro-Miguéns, D.G.; Vigo-Gendre, L.; López-Ares, D.; Freire-Garabal, M.; Núñez-Iglesias, M.J.; Novío, S. Crystals 2018, 8 (10), 369.
23. Coverdale, J.P.; Laroiya-McCarron, T.; Romero-Canelón, I. Inorganics 2019, 7 (3), 31.
24. Alessio, E.; Messori, L. Molecules 2019, 24 (10), 1995.
25. Sudhindra, P.; Sharma, S.A.; Roy, N.; Moharana, P.; Paira, P. Polyhedron 2020, 192, 114827.
26. Das, U.; Kar, B.; Pete, S.; Paira, P., Ru (II), Ir (III), Re (I) and Rh (III) based complexes as next generation anticancer metallopharmaceuticals. Dalton Transactions 2021, 50, 11249-11258.
27. Amarasinghe, T.U.; Perera, S.D. OUSL J. 2022, 17.
28. Mandal, P. Half-sandwich Ruthenium (II)-arene complexes as anticancer agents. Thesis, Department of Chemistry, IIT Indore, 2018.
29. Simović, A.R.; Masnikosa, R.; Bratsos, I.; Alessio, E. Coord. Chem. Rev. 2019, 398, 113011.
30. Cox, A.R.; Masschelin, P.M.; Saha, P.K.; Felix, J.B.; Sharp, R.; Lian, Z.; Xia, Y.; Chernis, N.; Bader, D.A.; Kim, K.H. Cell Metab. 2022, 34(12), 1932-1946.
31. Onodera, T.; Momose, I.; Kawada, M. Chem. Pharm. Bull. 2019, 67 (3), 186-191.
32. Sonzogni-Desautels, K.; Ndao, M. Front. Immunol. 2021, 12, 683694.
33. Chrysouli, M.; Banti, C.; Kourkoumelis, N.; Panayiotou, N.; Markopoulos, G.; Tasiopoulos, A.J.; Hadjikakou, S. J. Inorg. Biochem. 2018, 179, 107-120.
34. Odachowski, M.; Marschner, C.; Blom, B. Eur. J. Med. Chem. 2020, 204, 112613.
35. Fernández-Moreira, V.; Herrera, R.P.; Gimeno, M.C. Pure Appl. Chem. 2019, 91 (2), 247-269.
36. Mirzadeh, N.; Reddy, T.S.; Bhargava, S.K. Coord. Chem. Rev. 2019, 388, 343-359.
37. Peña, Q.; Wang, A.; Zaremba, O.; Shi, Y.; Scheeren, H.W.; Metselaar, J.M.; Kiessling, F.; Pallares, R.M.; Wuttke, S.; Lammers, T. Chem. Soc. Rev. 2022, 51 (7), 2544-2582.
38. Hoskins, C. Cancers 2020, 12 (8), 2127.
39. Zhan, W.; Wang, C.-H. J. Contr. Release 2018, 285, 212-229.
40. Machado, J.F.; Morais, T.S. Dalton Trans. 2022, 51 (7), 2593-2609.