Abstract
Per- and polyfluoroalkyl substances (PFAS) are a group of synthetic chemicals which have increasingly become a worldwide environmental and public health concern, including in Aotearoa. Known for their exceptional stability and resistance to degradation, PFAS have been widely used in industrial processes, consumer products, and firefighting foams. However, a number of adverse health effects have been linked to PFAS exposure, including increased risk of cancer.
History
PFAS are a diverse group of more than 10,000 man-made chemicals characterised by many carbon-fluorine bonds – one of the strongest covalent single bonds possible.1–3 This non-natural structure renders PFAS highly resistant to thermal and environmental degradation, leading to them often being called ‘forever chemicals’ within the media. The accidental discovery of marketable perfluorinated compounds began in the late 1930s at the DuPont chemical company where a researcher generated a tetrafluoroethylene resin while conducting experiments with Freon refrigerants (a class of refrigerant gasses composed of chlorofluorocarbons and hydrofluorocarbons).4 This resin, polytetrafluoroethylene (PTFE), was eventually commercialised and is now known as the low-friction, heat, and corrosion resistant material Teflon (Fig. 1).
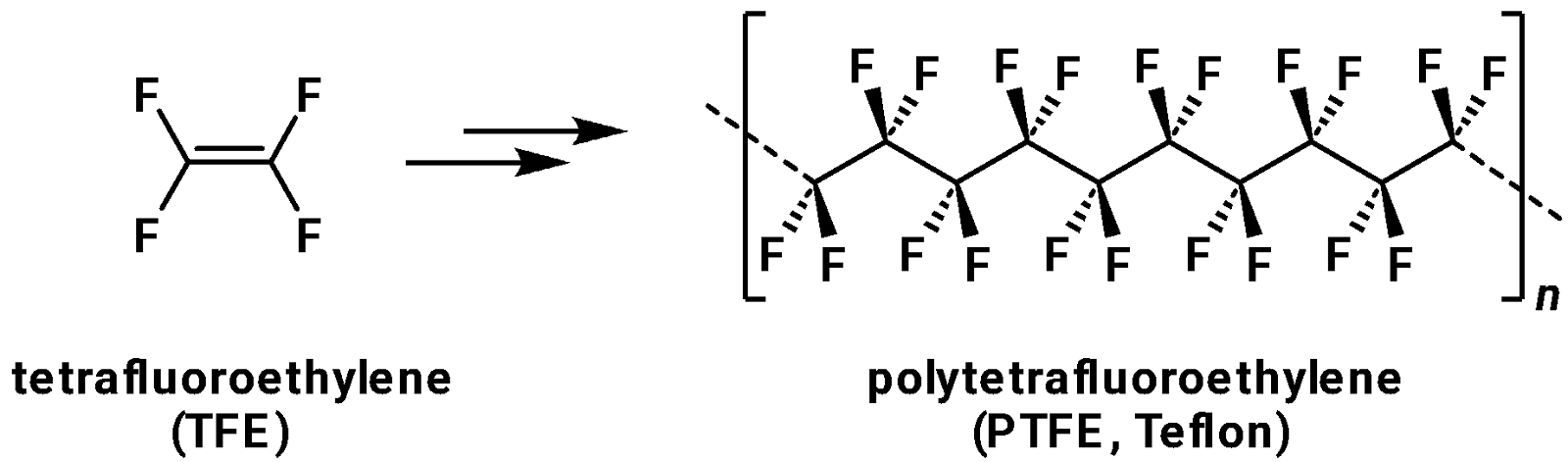
The widespread adoption of PFAS-based materials quickly expanded beyond Teflon into products like firefighting foams, waterproof textiles, non-stick cookware, and industrial lubricants. Their unique combination of high thermal stability, resistance to many solvents, and hydrophobicity have made them invaluable in a range of industries. However, the same stability that makes PFAS so useful also poses significant challenges for environmental and biological systems.5 PFAS have been detected in soil, water, and even living organisms worldwide, leading to growing concerns about their persistence, bioaccumulation, and potential toxicity.6–10 PFAS exposure has been linked to a variety of serious health issues, with carcinogenicity being one of the most concerning.11–15 Certain PFAS compounds have also been linked to other adverse health effects including liver damage and endocrine disruption, prompting regulatory efforts and ongoing research into safer alternatives.16–20
Following the discovery of PTFE by DuPont, 3M began the development of perfluorooctane sulfonates (PFOS), a key component of Scotchgard™, which became a hallmark product for stain and water repellent coatings in fabrics and textiles. PFOS and perfluorooctanoic acid (PFOA), another widely produced PFAS compound, became central to 3M’s fluorochemistry program. Similar to PTFE, PFOA and PFOS (Fig. 2) exhibit exceptional chemical and thermal stability, as well as the ability to repel water, oil, and grease when used as a material coating. 3M pioneered the electrochemical fluorination process used to synthesise PFOS, in which hydrocarbon compounds were exposed to anhydrous hydrogen fluoride (HF) gas and an electric current applied to promote the replacement of hydrogen atoms with fluorine.21 This method allowed the production of many different complex poly- and perfluorinated compounds at scale and formed the basis for 3M’s PFAS manufacturing. These fluorochemicals quickly found numerous applications, including in the textiles, automotive, and aviation industries. PFOS (and other PFAS) containing firefighting foams also became critical for their ability to extinguish oil and fuel fires.22

However, by the 1980s, internal research at 3M began highlighting the environmental persistence and potential health effects of these compounds (Fig. 3). Both PFOA and PFOS, two of the most extensively studied PFAS compounds, have been classified as possible human carcinogens by the International Agency for Research on Cancer (IARC).23 Despite these findings, production continued for several decades. Increasing regulatory pressure and growing public awareness in the early 2000s caused 3M to announce the voluntary phase-out of PFOS and PFOA, citing concerns about their environmental and biological persistence. This decision marked a significant turning point in the management of PFAS and spurred research into safer alternatives and sustainable manufacturing practices.4

Sources of PFAS contamination
PFAS contamination can originate from a variety of sources (Fig. 4). In Aotearoa, these can include firefighting foams, industrial discharge, landfills and wastewater treatment, and imported consumer products. For nearly five decades, aqueous film-forming foams (AFFFs) containing PFOS and PFOA were widely used in New Zealand for emergency response and firefighting training at airports, military bases, and industrial sites, with PFOS/PFOA containing foams in use by the New Zealand Defence Force up until 2018. These activities have left a legacy of PFAS contamination in soil and groundwater, particularly in the vicinity of Air Force bases where residual contamination remains an issue.24
Despite not producing PFAS on a large scale, New Zealand still faces environmental risks from these chemicals due to their presence in imported products like electronics, textiles, and metal plating materials. Everyday items such as non-stick cookware, stain-resistant carpets, water-repellent clothing, and food packaging are major contributors to PFAS pollution. During use and washing, these chemicals can leach into wastewater systems, eventually making their way into natural ecosystems. Additionally, PFAS-containing waste often ends up in landfills, while wastewater treatment plants struggle to remove these persistent substances. As a result, treated effluent and sludge may still harbour significant PFAS levels, potentially contaminating waterways or agricultural land when used as fertiliser.
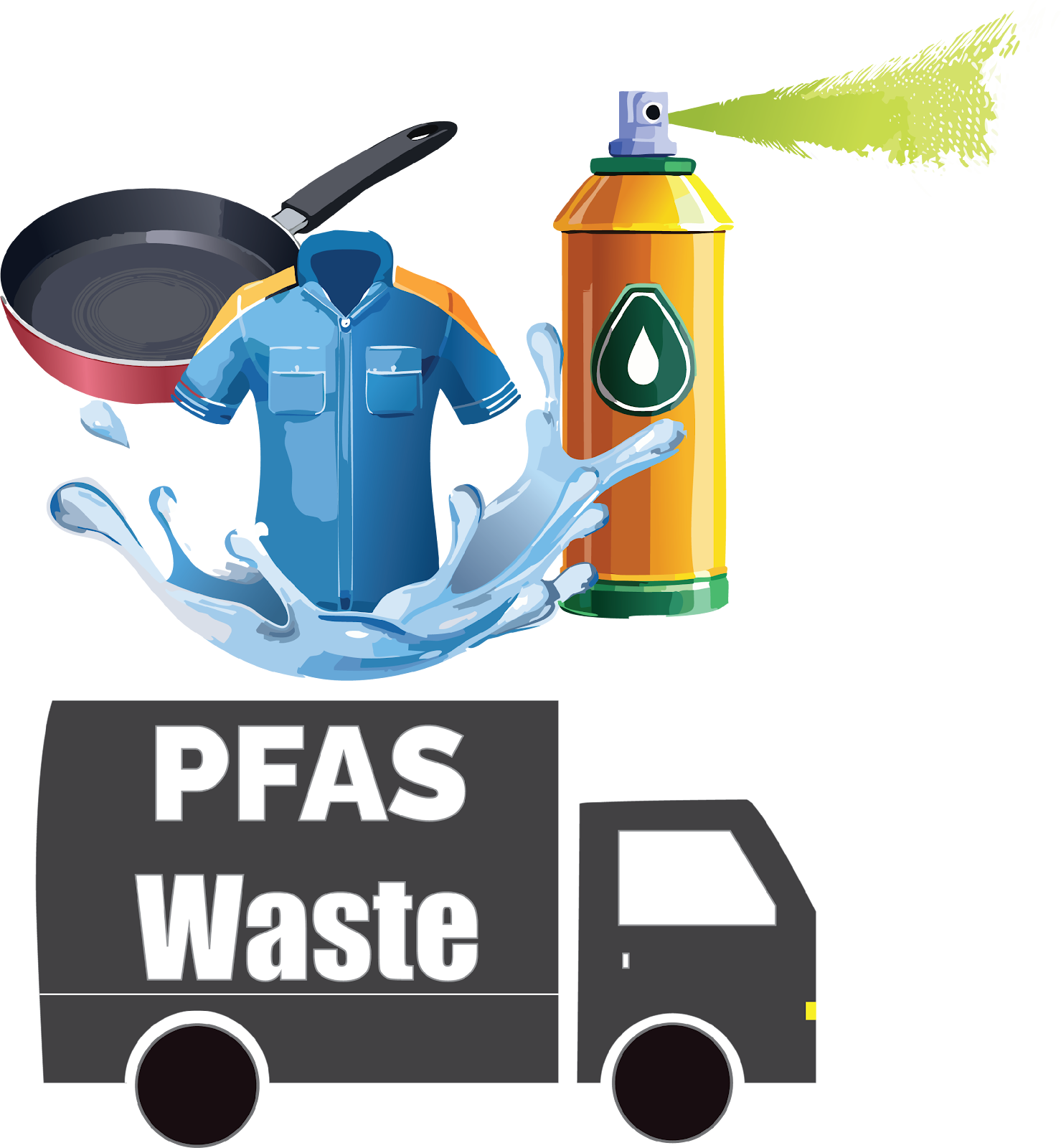
Prevalence of PFAS in New Zealand
PFAS contamination is a pressing environmental issue in New Zealand, with significant impacts documented across various sites, particularly where firefighting foams have been used extensively. Airports and military bases, such as Ohakea and Woodbourne, have been hotspots for PFAS pollution, with testing revealing elevated levels of these chemicals in both soil and groundwater samples.25,26 These incidents have highlighted the need for robust testing and risk management strategies, especially in areas with a history of intensive PFAS use.8,9,24
Drinking water contamination, though not comprehensively studied nationwide, has been identified in isolated cases, particularly in communities near firefighting training facilities. This raises concerns about the broader risks posed by PFAS exposure through water supplies, especially in the absence of consistent monitoring.8,9,24 Beyond freshwater systems, marine environments are also affected, with PFAS detected in coastal areas near urban and industrial zones.27 These contaminants have been found in both sediment and marine organisms, raising alarms about bioaccumulation in seafood and the potential carry-over risks to human and ecological health.28 Similar concerns relate to PFAS contaminated soil and water which infiltrates the agricultural sector, accumulating in crops and livestock and potentially entering the food chain — a risk well-documented internationally but less studied in New Zealand.29–33 Urban areas further contribute to PFAS contamination through stormwater runoff, which carries pollutants from roads, industrial facilities, and residential areas into rivers and coastal waters. This pervasiveness of PFAS underscores the urgent need for increased monitoring, targeted research, and effective regulatory measures. By identifying contamination sources and mitigating their impact, New Zealand can work towards protecting both public health and the environment from the long-term effects of these persistent pollutants.
Regulatory efforts in New Zealand
New Zealand has implemented several measures to address PFAS contamination, including the introduction of National Environmental Standards that set limits for PFAS levels in soil and water (Fig. 5). However, challenges persist in the consistent enforcement and application of these guidelines across different regions. To enhance these efforts, government agencies have initiated testing and monitoring programs at high-risk sites, such as industrial areas and locations near firefighting training facilities, to identify contamination hotspots and effectively guide remediation initiatives.
In line with international efforts under the Stockholm Convention, New Zealand has taken significant steps to phase out long-chain PFAS compounds, including PFOS and PFOA. The Environmental Protection Authority (EPA, Te Mana Rauhī Taiao) has imposed tighter restrictions on toxic and environmentally damaging firefighting foam products, with all PFAS-containing firefighting foams set to be completely banned by December 2025.34 Additionally, the EPA has banned the use of PFAS in cosmetic products from 31 December 2026, making New Zealand one of the first countries to take this step to protect people and the environment.35
Despite these advancements, addressing PFAS contamination in New Zealand remains an ongoing challenge. Legacy contamination from decades of industrial and agricultural use continues to impact ecosystems and communities. Moreover, the lack of enforceable limits for some newer PFAS compounds underscores the need for a proactive regulatory framework that evolves alongside scientific research. Enhancing public awareness campaigns and fostering collaboration between government agencies, industry stakeholders, and local communities will be crucial to effectively managing PFAS contamination and mitigating its long-term impacts.
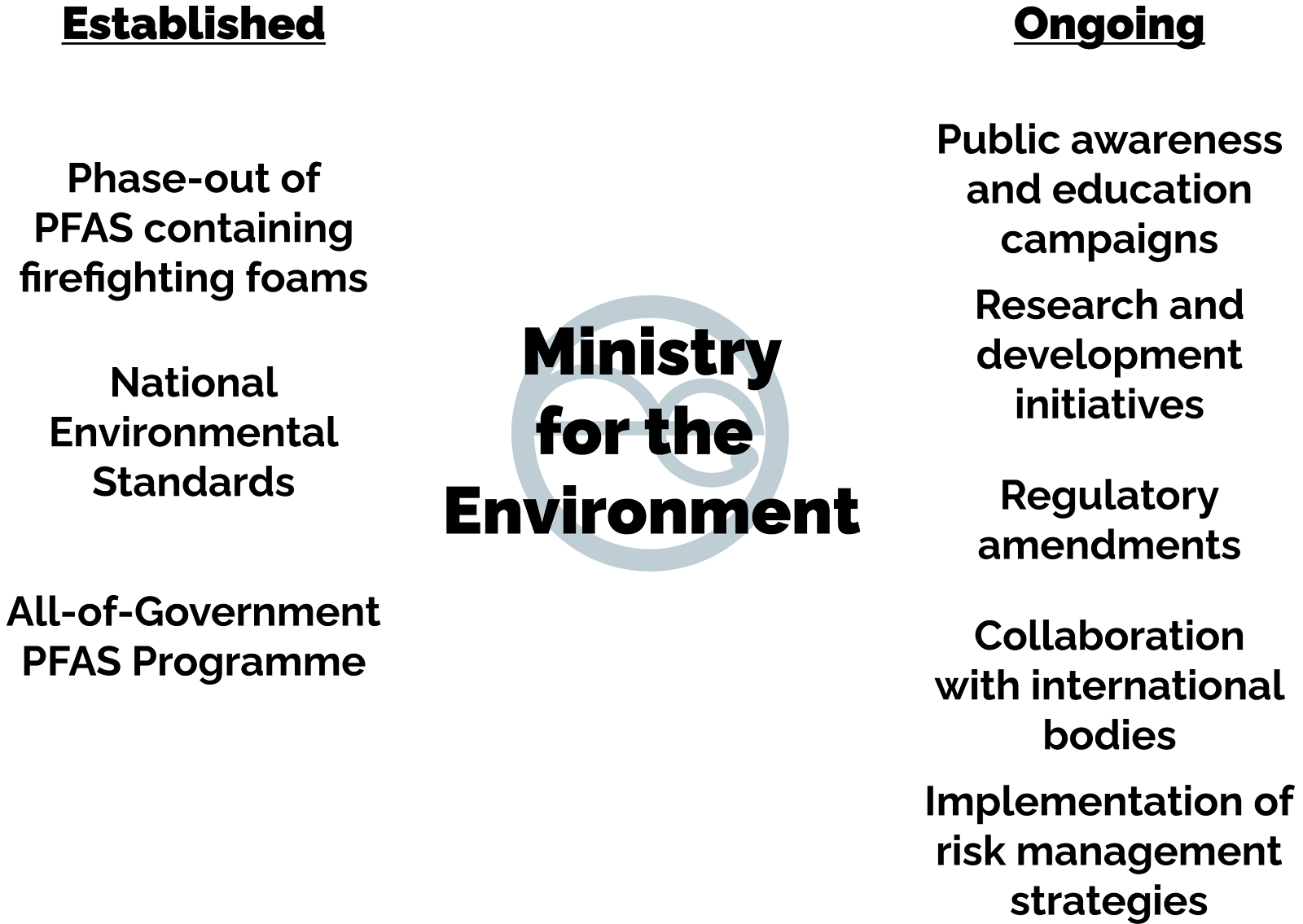
Remediation strategies
Given the persistence of per- and polyfluoroalkyl substances (PFAS) in the environment, effective remediation is both complex and costly. Current and emerging methods encompass a range of strategies aimed at containing, destroying, or removing these contaminants from affected sites. Each approach presents unique challenges and benefits, often necessitating a combination of methods tailored to specific contamination scenarios.
1. Containment and isolation
Containment and isolation strategies are designed to prevent the spread of PFAS from contaminated sites, thereby minimising exposure to surrounding environments and populations.
- Capping and lining: This method involves covering contaminated areas with impermeable materials, such as geomembranes or clay layers, to prevent water infiltration and subsequent PFAS migration. For example, capping can reduce the leaching of PFAS into groundwater in landfill settings. However, the long-term integrity of these barriers is crucial, as degradation over time can compromise their effectiveness.
- Pump-and-treat systems: This approach involves extracting contaminated groundwater, treating it to remove PFAS, and then safely discharging or reinjecting the purified water. While widely used, pump-and-treat systems are typically energy-intensive and may require extended operational periods to achieve the desired reduction in contaminant concentrations. Various studies have highlighted the challenges associated with this method, noting that the combination of high running costs and challenging chemical stability of PFAS often leads to short-lived projects or incomplete removal during treatment processes.36–38
2. Destruction technologies
Destruction technologies aim to break down PFAS compounds into less harmful substances, addressing the root of contamination rather than merely containing it.
- High-temperature incineration: PFAS can be thermally decomposed at temperatures exceeding 1,000°C. However, concerns have been raised regarding the potential formation of toxic byproducts and the complete destruction of PFAS during incineration. Research indicates that incomplete combustion can lead to the emission of hazardous substances, necessitating stringent controls and monitoring.39–41
- Electrochemical oxidation: This method utilises electrical currents to degrade PFAS molecules in contaminated water. Recent studies have demonstrated the effectiveness of electrochemical oxidation using low-cost graphene sponge electrodes, achieving significant removal efficiencies for various PFAS compounds. The process offers a promising avenue for PFAS remediation, though scalability and cost considerations remain under investigation.42–44
3. Filtration and adsorption
Filtration and adsorption techniques focus on removing PFAS from water through physical and chemical interactions, effectively reducing contaminant concentrations.
- Activated carbon: Granular activated carbon (GAC) is commonly employed to adsorb PFAS from water sources. While effective for certain PFAS compounds, the efficiency of GACs can vary, and it may require frequent replacement or regeneration. Studies have shown that GAC is more effective for long-chain PFAS, with reduced efficacy for shorter-chain variants.45,46
- Ion exchange resins: These synthetic materials are designed to selectively bind PFAS ions, facilitating their removal from water supplies. Research has demonstrated that ion exchange resins can achieve high removal efficiencies, particularly for short-chain PFAS, which are often challenging to capture using other methods. However, the spent resins require proper disposal or regeneration to prevent secondary contamination.47,48
- Membrane filtration: Techniques such as reverse osmosis and nanofiltration employ semi-permeable membranes to separate PFAS from water. These methods are highly effective, capable of removing a broad spectrum of PFAS compounds. Nonetheless, they generate concentrated waste streams that necessitate further treatment or disposal, posing additional environmental challenges.49,50
4. Emerging innovations
Innovative approaches are being explored to enhance PFAS remediation, focusing on developing more efficient and sustainable technologies.
- Bioremediation: This strategy investigates the potential of microorganisms to degrade PFAS compounds. Although traditional bioremediation has been limited due to PFAS's resistance to natural degradation, recent research is exploring genetically engineered microbes and specific environmental conditions that may enhance biodegradation pathways. For instance, certain bacteria have shown the ability to defluorinate PFAS under laboratory conditions, indicating a possible future application.51–53
- Photocatalysis: Utilising light-activated catalysts, this method aims to break down PFAS in contaminated water. Studies have identified specific photocatalytic materials capable of degrading PFAS when exposed to ultraviolet light, offering a potential low-energy solution for remediation. However, challenges remain in scaling up this technology and ensuring complete mineralisation of PFAS compounds.54–56
- Porous supramolecular materials: Porous supramolecular materials, such as metal-organic frameworks (MOFs), covalent organic frameworks (COFs), and metal-organic polyhedra (MOPs) are emerging as highly effective technologies for PFAS remediation due to their high surface areas, tunable pore structures, and versatile chemical properties. These materials can be engineered to selectively adsorb PFAS by incorporating functional groups designed to interact with the hydrophobic fluorocarbon chains or the polar head groups of PFAS compounds. For example, a tunable porous β-cyclodextrin (Fig. 6) polymer platform has demonstrated efficient removal of anionic PFAS at low adsorbent loadings, with different mechanisms for short- and long-chain PFAS removal.57,58

A particularly promising material is UiO-66 (Fig. 7), a zirconium-based MOF known for its stability and high adsorption efficiency. Studies have shown that introducing structural defects into UiO-66 enhances its adsorption capabilities for PFAS, such as perfluorooctane sulfonate and perfluorobutane sulfonate (PFBS), by increasing porosity and exposing additional active sites.59 Tailoring the pore size and surface chemistry of UiO-66 has further improved its performance in removing trace levels of PFAS, making it competitive with traditional adsorbents like activated carbon.60 Functionalising UiO-66 with amino groups has also been shown to enhance its hydrophobic interactions with PFAS, achieving adsorption capacities of up to 1653 mg/g for perfluorooctanoic acid.61 While porous supramolecular materials show considerable promise for PFAS remediation, challenges remain in terms of cost, scalability, and regeneration of the materials. Current research is focused on developing more affordable and sustainable production methods, improving reusability, and integrating these materials into existing water treatment systems to maximise their effectiveness in practical applications.
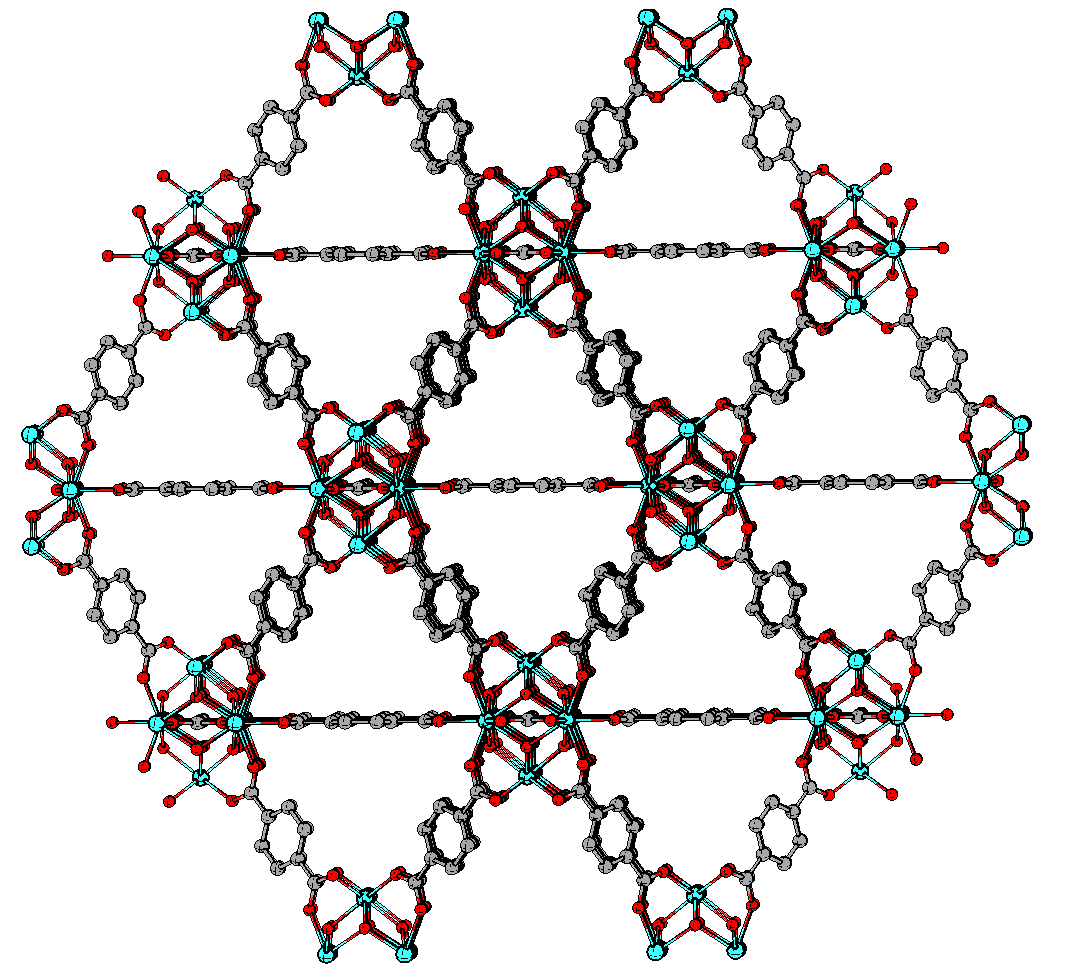
Each of these remediation strategies offers distinct advantages and limitations. The selection of an appropriate method depends on various factors, including the specific PFAS compounds present, site characteristics, and economic considerations. Often, a combination of approaches is employed to effectively address PFAS contamination, underscoring the need for continued research and development in this critical area.
Risk management strategies
In situations where complete remediation of PFAS contamination is not feasible, risk management strategies are essential to reduce exposure and protect human health and the environment. These approaches often involve restricting land use and providing alternative water supplies to affected communities. Restricting land use, for example, can help limit activities such as agriculture, construction, or residential development in contaminated areas, thereby reducing the risk of human exposure through soil contact, ingestion of contaminated produce, or water consumption. Local governments and environmental agencies can implement zoning regulations or issue site-specific advisories to ensure these restrictions are effectively communicated and enforced.
Providing alternative water supplies is one of the most direct and effective ways to protect affected populations, especially where PFAS contamination has infiltrated groundwater or public water systems. This strategy may involve delivering bottled water, drilling new wells into uncontaminated aquifers, or installing filtration systems like reverse osmosis units at homes or community facilities. Particularly in areas surrounding industrial sites or firefighting training facilities with known PFAS contamination, transitioning to community water systems equipped with granular activated carbon or ion exchange treatment technologies can significantly reduce exposure.
Public monitoring and education are also key components of effective risk management. Monitoring programs can track PFAS levels in water, soil, and air in contaminated areas to identify emerging risks and evaluate the effectiveness of protective measures. Public trust and education campaigns are equally critical, building relationships and informing communities about the risks associated with PFAS exposure and guiding them in adopting safer practices. For instance, educating the public on the consumption of fish from PFAS-contaminated water bodies or the use of private wells for drinking water can empower individuals to make well-informed decisions to protect their own health.
Although risk management does not address the contamination at its source, it serves as an essential interim measure, particularly in cases where PFAS levels are expected to persist for decades. Integrating risk management strategies with long-term remediation planning and investments in emerging technologies for PFAS removal or destruction is critical to achieving more sustainable solutions over time.
The way forward
Addressing PFAS contamination in New Zealand requires a coordinated and holistic approach to protect both human health and the environment. The persistence and widespread nature of PFAS demand immediate action across multiple fronts, including regulatory improvements, enhanced monitoring, investment in research, public education, and international collaboration. Together, these efforts can mitigate the risks posed by PFAS contamination while laying the groundwork for sustainable solutions. Strengthening regulations is a critical first step in addressing PFAS contamination. New Zealand currently lags behind Australia and America’s tightening regulation and limits on PFAS.62 Stricter standards must be adopted for PFAS levels in drinking water, soil, and food to align with international best practices and reflect the latest scientific findings. The EPA’s incoming ban on the use of PFAS in cosmetic products by December 2026 showcases leadership in regulating these harmful chemicals.34,35 Expanding this regulatory framework to limit PFAS emissions from industrial processes, phase out PFAS-containing products, and enforce contamination limits will be crucial for mitigating future risks.
Comprehensive and continuous monitoring programs are equally essential for managing PFAS contamination. Regular testing of water supplies, agricultural products, and high-risk sites is necessary to identify contamination hotspots and track trends over time. Groundwater testing by the EPA has revealed low levels of PFAS in some areas, suggesting a low immediate risk, but ongoing surveillance is needed to ensure early detection of emerging threats.63 A nationwide monitoring initiative would provide valuable data to guide mitigation strategies while fostering public trust in environmental management efforts. Investing in research and innovation is vital to developing cost-effective and sustainable remediation technologies for PFAS contamination. Scientific efforts must focus on understanding PFAS behaviour in the environment, improving removal methods, and exploring alternatives to PFAS-containing products. Collaborative initiatives, such as the All of Government PFAS Programme, emphasise the importance of coordinated research to inform system-wide improvements.24 Advances in technologies such as porous supramolecular materials, electrochemical oxidation, and bioremediation hold promise for transforming PFAS remediation in the future.
Transparent communication from government agencies will help build trust and encourage community engagement. At the same time, partnerships with other nations will enable New Zealand to share knowledge, adopt best practices, and contribute to global efforts in combating PFAS contamination. Engaging in international forums and agreements ensures that New Zealand remains at the forefront of PFAS management and remediation strategies. By prioritising these key actions, New Zealand can take meaningful steps to mitigate the impacts of PFAS contamination and protect its people and environment. Coordinated efforts across regulatory, scientific, and public domains will be essential to addressing this complex and persistent issue, ensuring long-term sustainability and resilience in the face of emerging environmental challenges.
Conclusions
Addressing PFAS contamination in New Zealand reflects global efforts to tackle these persistent pollutants. By strengthening regulations, enhancing monitoring, and investing in research, New Zealand can lead by example in protecting its environment and public health. From their industrial origins to their presence in everyday products, PFAS highlight the delicate balance between technological advancement and environmental responsibility. As a nation deeply connected to its natural landscapes and resources, New Zealand faces a unique imperative to safeguard its ecosystems and communities from the long-term risks associated with PFAS exposure.
Progress has been made through regulatory measures, public awareness, and the adoption of innovative remediation technologies. However, ongoing challenges, including legacy contamination and gaps in consistent monitoring, underscore the need for sustained action. Investing in research and fostering collaboration across government, industrial, and community sectors will be vital to developing more effective and sustainable solutions.
Looking ahead, the fight against PFAS contamination offers an opportunity for New Zealand to lead by example. By championing stringent regulations, advancing scientific understanding, and embracing international partnerships, the nation can demonstrate its commitment to environmental stewardship. Such efforts will not only mitigate the immediate impacts of PFAS but also pave the way for a cleaner, healthier future for generations to come.
References
- US EPA, O. PFAS Explained. https://www.epa.gov/pfas/pfas-explained (accessed 23/01/2025).
- PFAS & Their Uses | 3M PFAS. https://pfas.3m.com/pfas_uses (accessed 23/01/2025).
- The Science of Fluorochemistries | 3M PFAS. https://pfas.3m.com/the-science-of-fluorochemistries (accessed 23/01/2025).
- Magazine, S.; Molinek, R. The Long, Strange History of Teflon, the Indestructible Product Nothing Seems to Stick to. Smithsonian Magazine. https://www.smithsonianmag.com/science-nature/the-long-strange-history-of-teflon-the-indestructible-product-nothing-seems-to-stick-to-180984920/ (accessed 23/01/2025).
- Scientific Basis for Managing PFAS as a Chemical Class. https://pubs.acs.org/doi/10.1021/acs.estlett.0c00255?ref=pdf (accessed 23/01/2025.
- Wang, Z.; DeWitt, J. C.; Higgins, C. P.; Cousins, I. T. Environmental Science & Technology 2017, 51 (5), 2508–2518.
- Giesy, J. P.; Kannan, K. Environmental Science & Technology 2001, 35 (7), 1339–1342.
- Lenka, S. P.; Kah, M.; Padhye, L. P. Journal of Hazardous Materials 2022, 428, 128257.
- Buttle, E.; Sharp, E. L.; Fisher, K. New Zealand Geographer 2023, 79 (2), 97–106.
- DeWitt, J. C., Ed.; Toxicological Effects of Perfluoroalkyl and Polyfluoroalkyl Substances; Springer International Publishing: Cham, 2015.
- Zheng, J.; Liu, S.; Yang, J.; Zheng, S.; Sun, B. Science of The Total Environment 2024, 953, 176158.
- Temkin, A. M.; Hocevar, B. A.; Andrews, D. Q.; Naidenko, O. V.; Kamendulis, L. M. International Journal of Environmental Research and Public Health 2020, 17 (5), 1668.
- Pierozan, P.; Cattani, D.; Karlsson, O. Science of The Total Environment 2022, 808, 151945.
- Boyd, R. I.; Ahmad, S.; Singh, R.; Fazal, Z.; Prins, G. S.; Madak Erdogan, Z.; Irudayaraj, J.; Spinella, M. J. Cancers 2022, 14 (12), 2919.
- Steenland, K.; Winquist, A. Environmental Research 2021, 194, 110690.
- Sebe, G. O.; Anyaogu, E. V.; Ntomchukwu, A. D. A. R. C.; Oghenerhoro, S. O.; Jonathan, O. .E. Journal of Biosciences and Medicines 2023, 11 (12), 218–240.
- Solan, M. E.; Park, J.-A. Frontiers in Toxicology 2024, 6.
- Coperchini, F.; Awwad, O.; Rotondi, M.; Santini, F.; Imbriani, M.; Chiovato, L. Journal of Endocrinological Investigation 2017, 40 (2), 105–121.
- Costello, E.; Rock, S.; Stratakis, N.; Eckel, S. P.; Walker, D. I.; Valvi, D.; Cserbik, D.; Jenkins, T.; Xanthakos, S. A.; Kohli, R.; Sisley, S.; Vasiliou, V.; La Merrill, M. A.; Rosen, H.; Conti, D. V.; McConnell, R.; Chatzi, L. Environmental Health Perspectives 2022, 130 (4), 046001.
- IARC Monographs evaluate the carcinogenicity of perfluorooctanoic acid (PFOA) and perfluorooctanesulfonic acid (PFOS). https://www.iarc.who.int/news-events/iarc-monographs-evaluate-the-carcinogenicity-of-perfluorooctanoic-acid-pfoa-and-perfluorooctanesulfonic-acid-pfos (accessed 23/01/2025).
- 3M and Fluorochemicals | 3M India. https://www.3mindia.in/3M/en_IN/sustainability-in/annual-report/past-reports-policies/3m-and-fluorochemicals/ (accessed 23/01/2025).
- Forever chemicals - a lasting legacy? | Fire Protection Association. https://www.thefpa.co.uk/news/forever-chemicals-a-lasting-legacy- (accessed 23/01/2025).
- Zahm, S.; Bonde, J. P.; Chiu, W. A.; Hoppin, J.; Kanno, J.; Abdallah, M.; Blystone, C. R.; Calkins, M. M.; Dong, G.-H.; Dorman, D. C.; Fry, R.; Guo, H.; Haug, L. S.; Hofmann, J. N.; Iwasaki, M.; Machala, M.; Mancini, F. R.; Maria-Engler, S. S.; Møller, P.; Ng, J. C.; Pallardy, M.; Post, G. B.; Salihovic, S.; Schlezinger, J.; Soshilov, A.; Steenland, K.; Steffensen, I.-L.; Tryndyak, V.; White, A.; Woskie, S.; Fletcher, T.; Ahmadi, A.; Ahmadi, N.; Benbrahim-Tallaa, L.; Bijoux, W.; Chittiboyina, S.; de Conti, A.; Facchin, C.; Madia, F.; Mattock, H.; Merdas, M.; Pasqual, E.; Suonio, E.; Viegas, S.; Zupunski, L.; Wedekind, R.; Schubauer-Berigan, M. K. The Lancet Oncology 2024, 25 (1), 16–17.
- Close, M.; Banasiak, L. National Survey of Per- and Polyfluoroalkyl Substances (PFAS) in Groundwater 2022; Client Report CSC23006; Institute of Environmental Science and Research Limite, 2023. https://www.esr.cri.nz/media/dm3lccq4/esr-survey-pfas-contamination-groundwater-report-2023.pdf (accessed 24/01/2025).
- Rhodes, N. RNZAF Base Ohakea PFAS Investigation: Comprehensive Site Investigation Report; Client Report A02744805; Pattle Delamore Partners Ltd, 2019. https://environment.govt.nz/assets/what-government-is-doing/Land/Ohakea-CSIR-Part-A-2019.pdf.
- Walker, K. RNZAF Base Woodbourne PFAS Investigation: Comprehensive Site Investigation Report; Client Report C02150801; Pattle Delamore Partners Ltd, 2019. https://environment.govt.nz/assets/what-government-is-doing/Land/woodbourne-csir-2019-part-1.pdf.
- Walker, K. New Zealand Defence Force PFAS Investigation: Waitematā Harbour; Client Report A02684806; Pattle Delamore Partners Ltd, 2019. https://environment.govt.nz/assets/what-government-is-doing/Land/Waitemata-PFAS-CSIR-2019.pdf.
- Stockin, K. A.; Yi, S.; Northcott, G. L.; Betty, E. L.; Machovsky-Capuska, G. E.; Jones, B.; Perrott, M. R.; Law, R. J.; Rumsby, A.; Thelen, M. A.; Graham, L.; Palmer, E. I.; Tremblay, L. A. Marine Pollution Bulletin 2021, 173, 112896.
- Preisendanz, H. E.; Li, H.; Mashtare, M.; Mina, O. Journal of Environmental Quality 2025, 54 (1), 1–5.
- Jha, G.; Kankarla, V.; McLennon, E.; Pal, S.; Sihi, D.; Dari, B.; Diaz, D.; Nocco, M. International Journal of Environmental Research and Public Health 2021, 18 (23), 12550.
- Death, C.; Bell, C.; Champness, D.; Milne, C.; Reichman, S.; Hagen, T. Science of The Total Environment 2021, 774, 144795.
- Drew, R.; Hagen, T. G.; Champness, D. Food Additives & Contaminants: Part A 2021, 38 (11), 1897–1913.
- Rigby, H.; Dowding, A.; Fernandes, A.; Humphries, D.; Jones, N. R.; Lake, I.; Petch, R. G.; Reynolds, C. K.; Rose, M.; Smith, S. R. Science of The Total Environment 2021, 765, 142787.
- PFAS are forever – a complicated chemical family | EPA. https://www.epa.govt.nz/community-involvement/science-at-work/pfas/ (accessed 24/01/2025).
- EPA bans ‘forever chemicals’ in cosmetic products | EPA. https://www.epa.govt.nz/news-and-alerts/latest-news/epa-bans-forever-chemicals-in-cosmetic-products/ (accessed 24/01/2025).
- Birnstingl, J.; Wilson, J. Remediation Journal 2024, 35 (1), e70005.
- Ciampi, P.; Esposito, C.; Bartsch, E.; Alesi, E. J.; Petrangeli Papini, M. Environmental Research 2023, 234, 116538.
- Carroll, K. C.; Brusseau, M. L.; Tick, G. R.; Soltanian, M. R. Science of The Total Environment 2024, 918, 170600.
- K. Longendyke, G.; Katel, S.; Wang, Y. Environmental Science: Processes & Impacts 2022, 24 (2), 196–208.
- Weitz, K.; Kantner, D.; Kessler, A.; Key, H.; Larson, J.; Bodnar, W.; Parvathikar, S.; Davis, L.; Robey, N.; Taylor, P.; De la Cruz, F.; Tolaymat, T.; Weber, N.; Linak, W.; Krug, J.; Phelps, L. Science of The Total Environment 2024, 932, 172658.
- Strandberg, J.; Awad, R.; Bolinius, D. J.; Yang, J.-J.; Sandberg, J.; Bello, M. A.; Gobelius, L.; Egelrud, L.; Härnwall, E.-L. PFAS in Waste Residuals from Swedish Incineration Plants.
- Mirabediny, M.; Sun, J.; Yu, T. T.; Åkermark, B.; Das, B.; Kumar, N. Chemosphere 2023, 321, 138109.
- Veciana, M.; Bräunig, J.; Farhat, A.; Pype, M.-L.; Freguia, S.; Carvalho, G.; Keller, J.; Ledezma, P. Journal of Hazardous Materials 2022, 434, 128886.
- Sharma, S.; Shetti, N. P.; Basu, S.; Nadagouda, M. N.; Aminabhavi, T. M. Chemical Engineering Journal 2022, 430, 132895.
- Sonmez Baghirzade, B.; Zhang, Y.; Reuther, J. F.; Saleh, N. B.; Venkatesan, A. K.; Apul, O. G. Environmental Science & Technology 2021, 55 (9), 5608–5619.
- Gagliano, E.; Falciglia, P. P.; Zaker, Y.; Birben, N. C.; Karanfil, T.; Roccaro, P. Current Opinion in Chemical Engineering 2023, 42, 100955.
- Murray, C. C.; Marshall, R. E.; Liu, C. J.; Vatankhah, H.; Bellona, C. L. Journal of Water Process Engineering 2021, 44, 102342.
- Dixit, F.; Dutta, R.; Barbeau, B.; Berube, P.; Mohseni, M. Chemosphere 2021, 272, 129777.
- Das, S.; Ronen, A. Membranes 2022, 12 (7), 662.
- Lee, T.; Speth, T. F.; Nadagouda, M. N. Chemical Engineering Journal 2022, 431, 134023.
- Yi, S.; Shen, X.; Li, K.; Sun, B.; Olivares, C. I.; Men, Y.; Stockin, K. A.; Tremblay, L. A. Chapter 19 - Microbial Transformation of per- and Polyfluoroalkyl Substances. In Water Security: Big Data-Driven Risk Identification, Assessment and Control of Emerging Contaminants; Elsevier, 2024; pp 317–335.
- A. LaFond, J.; B. Hatzinger, P.; L. Guelfo, J.; Millerick, K.; Andrew Jackson, W. Environmental Science: Advances 2023, 2 (8), 1019–1041.
- Berhanu, A.; Mutanda, I.; Taolin, J.; Qaria, M. A.; Yang, B.; Zhu, D. Science of The Total Environment 2023, 859, 160010.
- Leonello, D.; Fendrich, M. A.; Parrino, F.; Patel, N.; Orlandi, M.; Miotello, A. Applied Sciences 2021, 11 (18), 8458.
- Zelekew, O. A.; Wang, Z.; Gu, Y.; Wu, Y. Journal of Environmental Chemical Engineering 2024, 12 (5), 113323.
- Xia, C.; Lim, X.; Yang, H.; Goodson, B. M.; Liu, J. Journal of Water Process Engineering 2022, 46, 102556.
- Wang, R.; Lin, Z.-W.; Klemes, M. J.; Ateia, M.; Trang, B.; Wang, J.; Ching, C.; Helbling, D. E.; Dichtel, W. R. ACS Central Science 2022, 8 (5), 663–669.
- Wacławek, S.; Krawczyk, K.; Silvestri, D.; Padil, V. V. T.; Řezanka, M.; Černík, M.; Jaroniec, M. Advances in Colloid and Interface Science 2022, 310, 102807.
- Clark, C. A.; Heck, K. N.; Powell, C. D.; Wong, M. S. ACS Sustainable Chemistry & Engineering 2019, 7 (7), 6619–6628.
- Ilić, N.; Tan, K.; Mayr, F.; Hou, S.; Aumeier, B. M.; Morales, E. M. C.; Hübner, U.; Cookman, J.; Schneemann, A.; Gagliardi, A.; Drewes, J. E.; Fischer, R. A.; Mukherjee, S. Advanced Materials 2413120.
- Ding, S.; Zeng, X. arXiv September 25, 2021. https://doi.org/10.48550/arXiv.2109.12435.
- Saturday; November 2024, 2; RNZ, 5:44 pm Article: Australia, US Slash Limits On “Forever Chemicals” In Drinking Water, But NZ Staying Put | Scoop News. https://www.scoop.co.nz/stories/GE2411/S00006/australia-us-slash-limits-on-forever-chemicals-in-drinking-water-but-nz-staying-put.htm (accessed 27/01/2025).
- EPA releases findings on forever chemicals in groundwater | EPA. https://www.epa.govt.nz/news-and-alerts/latest-news/epa-releases-findings-on-forever-chemicals-in-groundwater/ (accessed 27/01/2025).